Research Description for Non-Scientists
Introduction
The Chun Lab’s research was born from a longstanding interest in understanding how the brain, particularly the cerebral cortex, develops. Over decades, this line of inquiry gave rise to two novel fields of research that form the core of our current efforts: 1) the actions of small fat molecules that are known as “lysophospholipids” that act as chemical signals through sensors on cells that are known as receptors; and 2) the surprising organization of the brain as an intricate mosaic of cells that have distinct DNA – distinct genomes – amongst cells from the same person. These basic discoveries have led to insights for both the normal brain and to diseases that afflict it.
Good Fat: Lysophospholipids (LPs)
The first field encompasses our efforts to understand the biological functions of certain fat derivatives, called lysophospholipids (LPs). Fats are often shunned in popular culture, and yet it is remarkable that our brains consist mostly of fat – lipids – when considered as a percentage of its dry weight (i.e., if one were to remove water from the brain, the next most abundant material are lipids). The fat you ingest includes triglycerides that, as the name implies, contain three attached fatty acid chains. Triglycerides can be absorbed by your body and broken down to release free fatty acids. These free fatty acids are used to build the phospholipids that make up the cell membrane of every cell in the body. Some phospholipids can be metabolized into LPs that contain only one chain. The breakdown and formation of all these lipid molecules is a dynamic process that is constantly altering the cellular environment in response to external stimuli. Two examples of LPs are “lysophosphatidic acid (LPA)” and sphingosine 1-phosphate (S1P), which are part of a larger family of other LPs.
A key discovery was the identification of specific receptors for LPs, and there are now six LPA receptors, five S1P receptors, and likely more that mediate the effects of other LPs.
Hydrocephalus
Accumulation of water in the brain, producing disturbingly enlarged heads, is perhaps the most extremely obvious neurological disease. Hydrocephalus that originates and manifests early in life has been reported to be the most common neurological disease of this age group. Hydrocephalus has historically been thought to be a “plumbing” problem, with the blockage of fluid outflow and the over-accumulation of fluid (cerebral spinal fluid) in the brain. Some clues on what can lead to hydrocephalus have come from the clinical literature, including fetal exposure to blood in the brain. LPA can be a major component of blood, especially after hemorrhage (e.g., during formation of blood clots). Too much LPA, acting through its receptors on immature brain cells, was found to profoundly disrupt the normal development of the brain before birth, which resulted in hydrocephalus (Figure 1).
Currently, only surgical, palliative treatments exist for treating hydrocephalus. The involvement of defined LPA receptors in this disease could offer a new, medical way to treat or prevent hydrocephalus, and studies to understand both the mechanism and LPA-relevant therapies are being pursued.
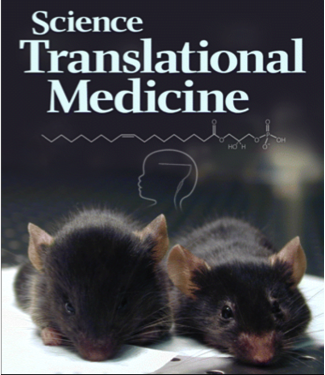
Figure 1. LPA (lysophosphatidic acid) signaling in hydrocephalus (Sci Transl Med 2011;3:99ra87, 2011).
Multiple Sclerosis (MS)
MS is the most common cause of neurological disability in young adults. It is a disease in which the body’s immune system attacks the brain and it produces a range of neurodegenerative symptoms that effect function and leads ultimately to death. Common treatments had historically involved shots or injections. In 2010, the FDA approved a compound known as fingolimod (commercial name Gilenya) that represented the first oral therapy for MS. Fingolimod was discovered in Japan during efforts to understand and modify chemicals produced by fungi; one species of fungus called Isaria sinclairii – a curious fungus that infects and grows out of the body of underground insects (Figure 2) – led to the identification of fingolimod. This phylum of fungi that includes Isaria sinclairii (called Ascomycota) is known in Asian herbal medicine as “winter worm, summer grass” because of this curious life-cycle.
Fingolimod turns out to be a precursor molecule that upon modification, becomes an S1P receptor modulator. How individual receptors affect different cell types is still unclear; while initial results showed that fingolimond acts in an anti-inflammatory fashion, work from multiple labs has shown that it works directly on cells of the brain. Current studies are focused on understanding this direct brain activity and its ability to prevent or repair damage caused by MS in the brain. Genetically altered mice and cutting-edge cellular and molecular approaches are being used to approach this project.
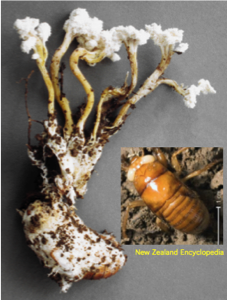
Figure 2. The fungus Isaria sinclairii, which grows out of insects, led to fingolimod. Studies in Japan on chemically modified natural products led to the identification of fingolimod. (Discov Med 2012;12:213-228).
Genomic Mosaicism: Your brain with myriad computer chips
Imagine that your brain is a supercomputer that contains billions of identical microchips. In this analogy, each chip would represent a neuron, with equivalent potential to process information on every chip to allow your computer – your brain – to function.
What would happen to the computer if you could actually customize each chip, altering the “hardwired” information on each chip in assembling an individual computer? Some chips could have more capacity or could be streamlined to eliminate redundancy, or perhaps customized in ways to improve speed, conserve energy, avoid viruses, and so forth. This analogy – admittedly imperfect – captures some of what appears to be occurring during the formation of brain, whereby individual neurons can vary at the level of their individual genome, resulting in brains that are unique mosaics of cells with different combinations of distinct genomes.
The human brain contains an enormously diverse repertoire of cells based on their appearance, molecular profile, and function. The vast majority of these cells arise before birth (e.g., neurons of the cerebral cortex) or soon after birth, thus “gifting” a person with neurons that will hopefully remain throughout life. Cells in the developing brain divide very rapidly. During cell division, DNA is replicated then distributed equally into each daughter cell. The cell packages DNA into chromosomes, discrete clumps of the genome that can be seen with a microscope and are characteristic in number or form for a given species. Human cells have 23 pairs of chromosomes (46 chromosomes of which two are sex chromosomes), one copy containing your mom’s genes and the other from your dad. Each chromosome contains hundreds of genes along with even more “non-coding” DNA that control the expression of all the components that make up cells, tissues, and organs of the body.
During studies of the brain, and genes that could affect DNA, we observed that sometimes the chromosomes are not equally distributed when cells in the developing brain divide. This results in cells with less than or more than two copies of each chromosome; we call these aneuploid cells. Aneuploid cells are found outside of the brain, most notably in cancer cells. Textbooks continue to teach the idea that every human cell must have 23 pairs of chromosomes – no more, no less – in order to function normally. Cells that have lost or gained chromosomes are presumed to either die or become cancerous. However, we have found that aneuploid cells are produced in high numbers in the developing brain – perhaps upwards of 40% – and although many of these cells do die, many of them also appear to survive to both influence development and to become part of the adult brain (Figure 3). This phenomenon appears to occur in all vertebrates.

Figure 3. Spectral karyotyping identifies aneuploid neural progenitor cells from the embryonic brain. Two extreme examples showing coincident gain and loss (A) and complete loss (B; called a nullisomy) are noted. PNAS 2001;98:13361-13366, J Neurosci 2012;32:16213-16222).
The existence of aneuploidy is also a clue that other processes affecting the genome are taking place (this is called genome instability) and research has revealed that other forms of changes to the genome are taking place in brain cells. We speculate that aneuploidy and other forms of variation contribute to the diversity of brain cells, allowing the brain to perform highly complex functions such as learning, memory, and creative thought, and contributing to human uniqueness, ranging from genius to madness. In addition, the myriad diseases afflicting the human brain are notable for being overwhelmingly sporadic in nature: this contrasts with explanations for diseases being due purely to genetics, wherein families with a “bad” gene pass the gene on to their affected children, and to every cell of that child. Modern techniques are now being used to understand how the genome changes, what produces the change, and the consequences of that change in the normal as well as diseased brain.
Our Lab
Our Home Page
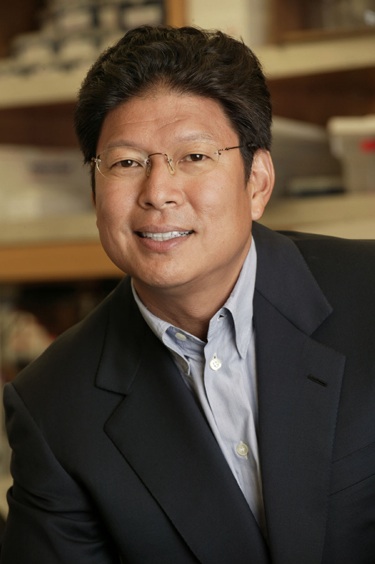
Visit our research page for scientists
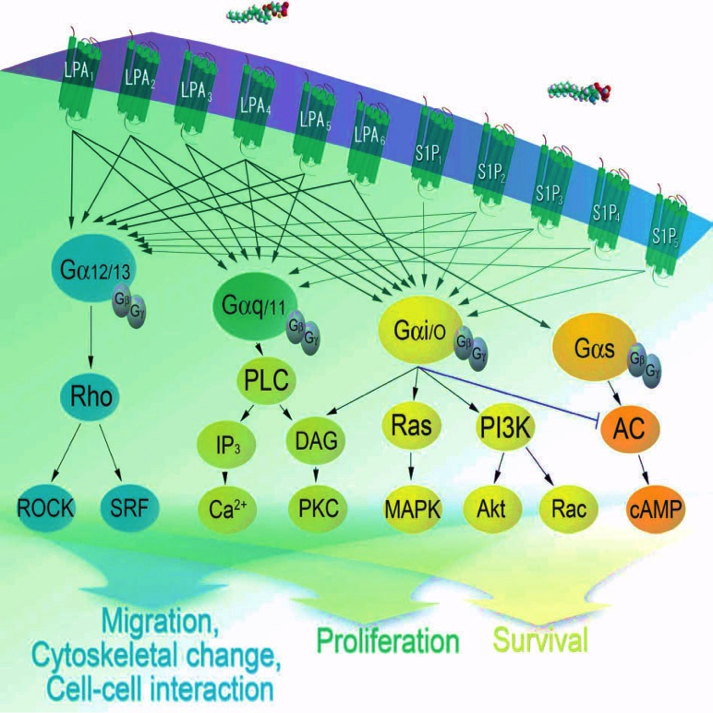
Visit our research page for non-scientists
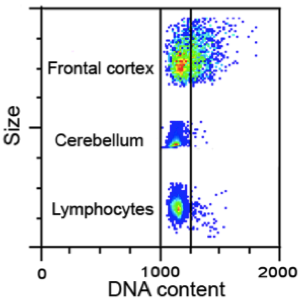
Visit our publications page
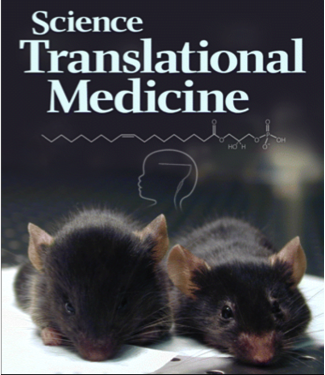
Our lab members
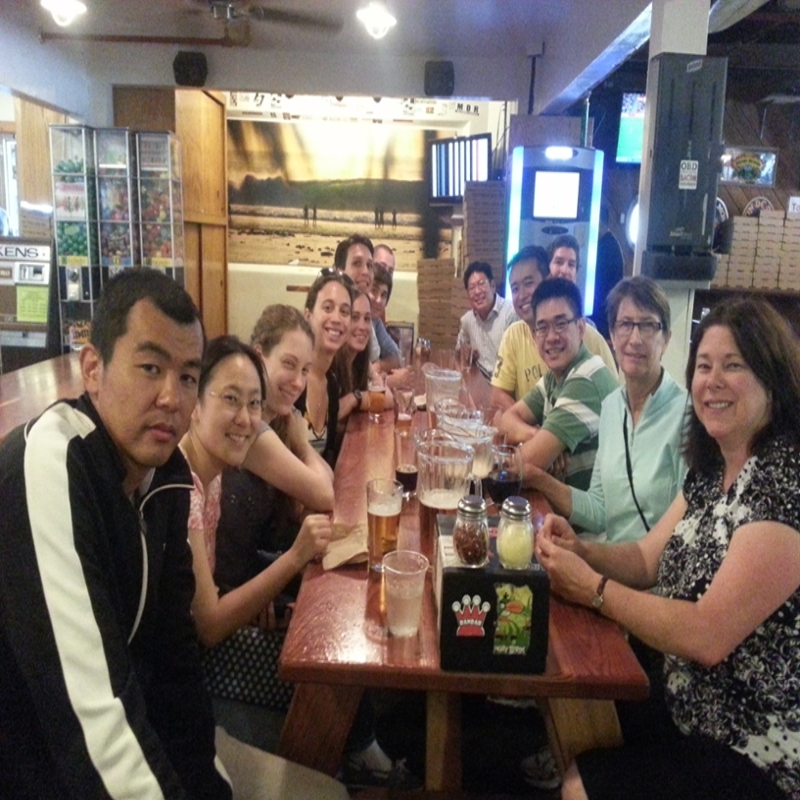
Want to see more photos of us? Click here!
Click here to see more information on how to contact our lab.
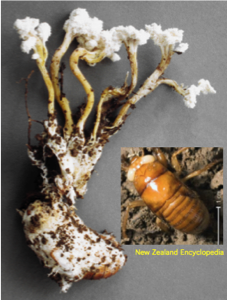