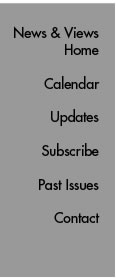 |
 |
On Genetics:
An Interview with Bruce Beutler
Bruce Beutler, chair of a newly created department at The Scripps Research Institute, the Department of Genetics, speaks with Mika Ono of News&Views about the new department, progress in the field of genetics, and the direction of his own work.
News&Views: Tell me about your vision for the new Department of Genetics.
Beutler: Every new department has a story behind it. Probably most often, it's the chairman's story. I've worked throughout my career in the field of immunology, and until now, have been in the Department of Immunology at Scripps. So the biological problems that I have addressed have revolved around how we defend ourselves from infection. But along the way, partly by chance and partly by necessity, I became a geneticist. There are many ways to practice genetics. Different geneticists use different organisms and different analytical tools. And of course they study different questions about how living systems work. But genetics has a unifying philosophy: one that is free from bias, does not depend upon guesswork, and has solved some of the deepest mysteries in biology.
I realized how enormously powerful genetic methods can be by using genetics to understand how we sense infections: how we "know" we are sick. And given this background, I am going to build a department that will use genetics on a large scale to tell us much more about resistance to infection. We will learn how resistance works, and how it might be made to work even better. If you want to use genetics to solve a large problem, it's hard to pick a better one than infectious disease. Infection has made our genome the way it is.
How do we know that?
Heritability of resistance to infection has been formally measured in humans. In a classic study done close to 20 years ago, T.I. Sorensen looked at 960 Danish children who were adopted away from their biological parents. He followed these children over a period of 60 years retrospectively, looking at all causes of mortality. He also looked at the biological parents and tracked their mortality. Sorensen showed that if you have a biological parent who dies before the age of 50, you yourself are also likely to die prematurely. So, premature death is heritable. And when premature death is categorized by cause, there's no cause of death more heritable than infection.
That's interesting, because infection is usually thought of as environmental!
Exactly right. What could be more environmental than infection? But resistance to infection is genetic, of course, and the fact that it's so highly heritable says there must be a huge number of genes required for resistance. Furthermore, infection is a tremendous selective pressure for our own species, and probably for most life forms. About one-quarter of all people born today will ultimately die of infection. The same can be said of cancer, but the difference is that most people who die of cancer die at an advanced age. So susceptibility to cancer doesn't often influence whether a person will transmit his or her genes to the next generation. In contrast, most people who die of infection die in childhood. If you don't have strong resistance to infection, it is likely that you will leave no heirs. The selective pressure of infections was probably even greater in the recent past, before the introduction of public health measures and antibiotics.
If you had your pick, what kind of science would you add to your department at this point?
I would like the department to use a wide variety of genetic tools, and as much as possible, leverage the outstanding scientific strengths of Scripps as a whole to address a well defined range of topics. In my own lab, we use forward genetics, and apply it at the level of the germ line. That is, we make mutations at random in germ cells, and then find the genetic cause of each particular phenotype [physical characteristics of an individual] that we detect though screening. However, there are others who practice reverse genetics with great skill. They make hypotheses and then test them by observing the effects of mutations that they create in particular genes of their own choosing. There is also a role for in vitro genetics. In other words, one can use mutagens like transposons or retroviruses to disrupt genes in cultured cells at random, isolate cells with a particular trait, and then go back to find out what genes were disrupted. This can be a rapid and relatively inexpensive way to look for genes that operate at the level of individual cells: for example, some of those that are that required to cope with a viral infection. We want to incorporate all of these approaches into a strong Department of Genetics, while maintaining focus, at least in the beginning, on the large issue of host resistance to infection.
Beyond this, I foresee close interactions with the Departments of Immunology, Chemistry, Molecular Biology, and Cell Biology. Interaction with Immunology is just common sense. We are both interested in host resistance and speak the same language. Chemistry is already beginning to give us new phenomena to study. For instance, when a drug activates cytokine production by an unknown mechanism, how better to find the mechanism than by creating mutations that cancel the effect of the drug, and then, use genetics to find the protein that was required? And in the Departments of Molecular Biology and Cell Biology, we hope to collaborate with some of the most gifted structural biologists and cell biologists in the world, who may teach us exactly how the proteins we identify do what they do.
Could you tell me more about the differences between reverse and forward genetics?
Sure. Forward genetics is phenotype-driven. We look at the biological effects of thousands or even millions of random mutations induced by chemical mutagens and pick the mutations that cause the exact effects we are interested in. Let us suppose, for example, that a certain mutation makes a mouse become ill when it develops an infection that would ordinarily be trivial, even unnoticeable. We then find out what gene was been hit—the one that normally enables a mouse to be resistant to the infection. When we find it, we know with certainty that we have found a component of our immune armamentarium. We may not know how the component works, but we know it is essential. In contrast, reverse genetics starts with a gene. The gene may look interesting for some reason. For example, the protein it encodes might contain motifs that make it look like an immune signaling protein of some kind. The reverse geneticist says, "I wonder what this protein does." He or she may then target the gene, knocking it out in order to find out what its protein product does by observing the effect of its absence. Both of these approaches are powerful and important.
I would say that of the two methods, forward genetics tends to create more surprise, while reverse genetics can be more rapid and efficient. Those of us who practice forward genetics spend about half of our time creating questions, and half of our time answering them. A strange new phenotype can break open whole new fields. One may see a phenotype that nobody ever would have thought possible, and this has happened on many occasions. For example, long ago, other workers found strain of mouse that couldn't respond to endotoxin, a highly inflammatory molecule made by certain bacteria. Right away, it could be deduced that mice must have a non-redundant pathway for endotoxin sensing. That knowledge was really the start of much of our own work—and it was an out-of-the-blue surprise. You see a new phenotype, and then you have a new puzzle to solve. Reverse genetics is different. You have a hypothesis. You might have 10 genes that you suspect are involved in a certain process. You knock them all out, and you may find that you were right, or that you were wrong. But you will answer the question quickly.
Now, a by-product of the forward genetics in our department is certainly going to be the creation of developmental phenotypes, behavioral phenotypes, metabolic phenotypes, and other phenotypes that could be useful to scientists with interests distinct from our own. Indeed, I hope that other researchers at Scripps will take advantage of the opportunities. Already we've had collaborations with groups who are just better placed than we are to understand mice with behavioral anomalies, and mice that develop abnormally.
How do you get the word out to scientists who might be interested in the models you generate?
We have a website: mutagenetix.scripps.edu. There, you can find an early version of our catalog of mutations, frequently asked questions about our approach, information on how we map mutations, and more. We'd absolutely love to have other researchers pick up on some of these models. In fact, one of our biggest problems is to write about the mutations and distribute them as rapidly as they are discovered.
What do you see as the major challenges in the field of genetics right now?
It's really the golden age of genetics now. The public perception is that the genome was sequenced to solve all kinds of health problems, like cancer, aging, and diabetes, and this is true. But the means to this end is the acceleration of positional cloning: the method used by forward geneticists in their search for mutations. A decade ago, finding a mutation was a tremendous effort. A lab often had to sequence millions of base pairs of DNA in order to succeed. Nowadays, if we have a phenotype and we do a certain amount of mapping, we ourselves don't have to sequence a huge interval of DNA to find the gene that might be "the one." It's all been done for us. Instead, we go to the Web and look up what genes are present, knowing that the mutation must be in one of them. And we sequence the candidates in the mutant, which is a far easier task.
Times have changed.
Times certainly have changed. The genome has now been sequenced in the human, the mouse, the fruit fly, and other species of interest to geneticists. And sequencing technology itself is advancing very, very rapidly, so it has become relatively easy to find the cause of a phenotype. In fact, one large frontier in genetics concerns sequencing technology—making it work even better and faster. Many of us look forward to the time that a whole mammalian genome will be sequenced for $1,000, which has been posed as a near-term challenge. Perhaps one day it will cost even less, perhaps a dollar. One day it may be that I will take a few blood cells from a mouse or a person, and in an hour, know the whole sequence of the genome. At that point, we might see a funny looking mouse in the morning, and declare by afternoon, "This phenotype is caused by this amino acid change in such-and-such gene." No genetic mapping; no need to eliminate other possibilities; just pure cause and effect. You could even say that when we achieve such capabilities, we will have reached the "end of genetics." Then the new frontier might be epigenetics—the chemical changes that enforce gene regulation. Each of us begins life as a zygote, and the zygote divides again and again, but eventually we wind up with liver cells, brain cells, heart cells, lymphocytes, and so on. These all have the same genetic material, yet they look different, and have very different functions. How were they programmed to do what they do? That is not exactly a genetic question, but an epigenetic one.
So now that the genome has been sequenced, at this point you're figuring out what that means.
Yes. Stage one was sequencing the genome of humans and of all the model organisms we study. Stage two, now in progress, is finding what every gene does. Stage three, also in progress but a bit closer to the starting gate, is understanding how all genes are regulated. Those are the great challenges in genetics in the post-genomic world.
While we're focused on one category of genes—genes that cause resistance to infection—it turns out that a lot of these genes also do other things. For example, we find that mice with a grey coat instead of the normal black coat seen in the strain that we use. These hypopigmented mice often have immunodeficiencies. The reason for the immunodeficiency is that their immune cells, such as neutrophils and NK cells, cannot secrete granules properly. The hypopigmentation comes about because their melanocytes cannot secrete granules of pigment to the hair shaft. Different biological systems use the same building blocks to accomplish different ends, so it's not uncommon that immunocompromising mutations also have developmental, neurological, or pigmentation effects. Many of the genes required to resist an infection are multifunctional.
What direction is your lab going in right now?
My own laboratory is focusing on two areas: Toll-like receptor (TLR) signaling and resistance to viral infection. These areas are related because some mutations that interfere with TLR signaling can cause impressive susceptibility to viral infections, and for that matter, to other kinds of infection as well. However, we want to look broadly at everything required for surviving a viral infection. We actually run a screen in two directions. We look for mice that have trouble when they sustain a trivial infection, as well as mice that survive viral infection exceptionally well. Mice of the latter type are especially exciting. It seems to us that some of our proteins are not required for us to live, but clearly are required for progression of a viral infection. Such proteins might be targeted by a fundamentally new class of anti-viral drugs.
What I'm describing can be thought of as "latent" immune functions that have never evolved, but are revealed by our mutagen. Many proteins that mediate host resistance are very "broad-spectrum." In other words, some individual proteins that we use to fight infection make us resistant to viruses, bacteria, protozoa, and fungi, even though these microbes are very different from one another. It may be that the mutations we're introducing will confer resistance to many different microbes, and by targeting the corresponding proteins with drugs, we may have a similar effect. At least that is one possible outcome. Even if we could find host-oriented drugs that could make humans resistant to just one kind of microbe, it would be very rewarding.
Has any of your own work led to therapeutics like you're describing?
Well, in the past, certainly. Look behind you. There's a patent on the wall. That was issued for an inhibitor of TNF [tumor necrosis factor] that I designed. My present work really began with the isolation of TNF, and with the identification of TNF as an inflammatory mediator. Today, this TNF inhibitor is used to treat rheumatoid arthritis, as a drug called Enbrel. As for the mutations I've been telling you about now, the ones that affect the sensing of infection and are required for host resistance? Not yet…
It's a long path from the lab to the clinic.
It is a long way, yes. But I do think that some of the mutations we're finding now are going to be the basis of important therapeutics. It's a bit like gambling. Some days, we may find nothing at all. But every morning we can hope to see another fantastic mutation that will tell us how host resistance works, how it can fail, and even why it is that the host attacks sometimes itself.
Send comments to: mikaono[at]scripps.edu
|
 |
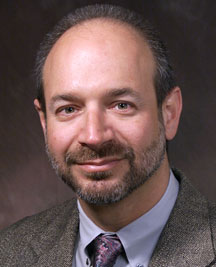
Bruce Beutler chairs a new Scripps Research department, the Department of Genetics.
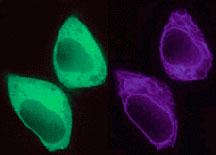
The Beutler lab focuses on investigating toll-like receptor (TLR) signaling and resistance to viral infection. Click for image details.
|
 |